Brandeis Alumni, Family and Friends
The Amber of Our Thoughts
December 15, 2022
How are memories created and preserved? Brandeis scientists are studying the brain to find out — and, ultimately, untangle disorders like Alzheimer’s and dementia.
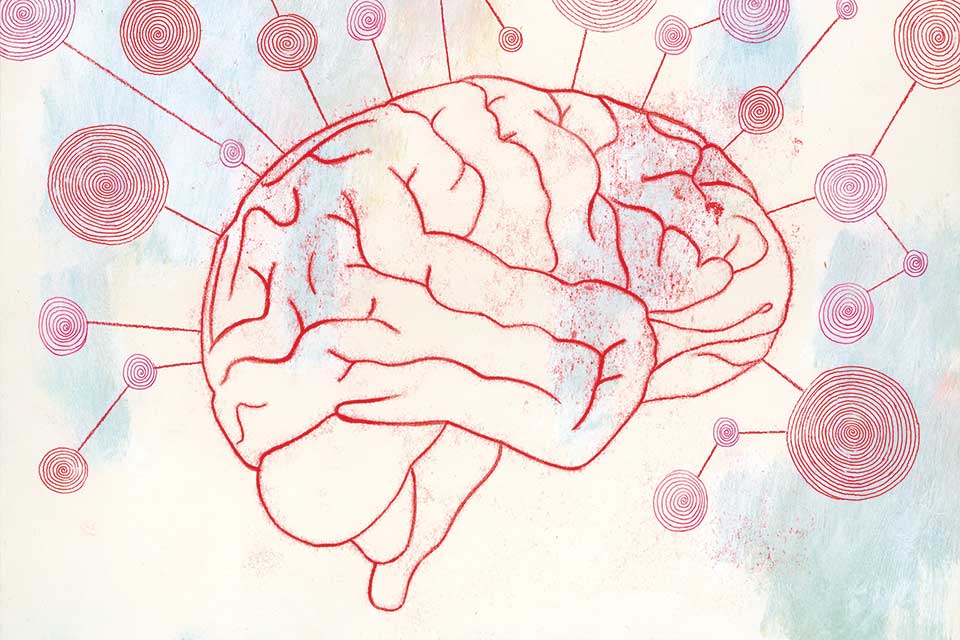
It was dinnertime in Hartford, Connecticut. As the sun dipped toward the horizon, casting long midsummer shadows over the city, a young boy hurrying home stepped off the curb alongside a busy street. He never saw the bicycle that hit him.
When 7-year-old Henry Molaison came to a few minutes later, his life had changed forever. Seizures began to grip his body at random, growing more intense with each passing year. By his mid-20s, these episodes had become so frequent and extreme he could no longer hold a job or lead a semblance of a normal life.
Desperate for relief, Molaison in 1953 agreed to a controversial brain surgery called a lobotomy, which would remove two small sections from the center of his brain. Doctors hoped the surgery would cure his crippling problems. It did, to a degree — the seizures disappeared. Unfortunately, they were replaced with a horrifying and unintended side effect: Molaison could no longer form new memories. Until his death in 2008, every experience he had after the surgery — every conversation, interaction, and emotion — evaporated from his mind like a strange dream.
This unusual trait transformed Molaison into Patient H.M., one of the most famous cases in the history of neuroscience. By studying his condition, researchers have learned a vast amount about how the brain works. And his fate raised some deeply existential questions: What, exactly, are our memories? How do they form and thrive in our brains? And if they suddenly vanish, what’s left of us?
Brandeis scientists are attempting to answer these questions, which philosophers and theologians have debated for centuries, by literally probing the brain, studying individual cells embedded within its gray matter, trying to understand at a biological level how a memory forms. Eventually, this basic research could lead to new classes of treatments for diseases of memory, like Alzheimer’s and dementia.
The mechanisms these scientists are discovering have implications far beyond medicine, however. They’re not only providing insights into the brain as an organ, they’re helping unravel the biology of consciousness itself.
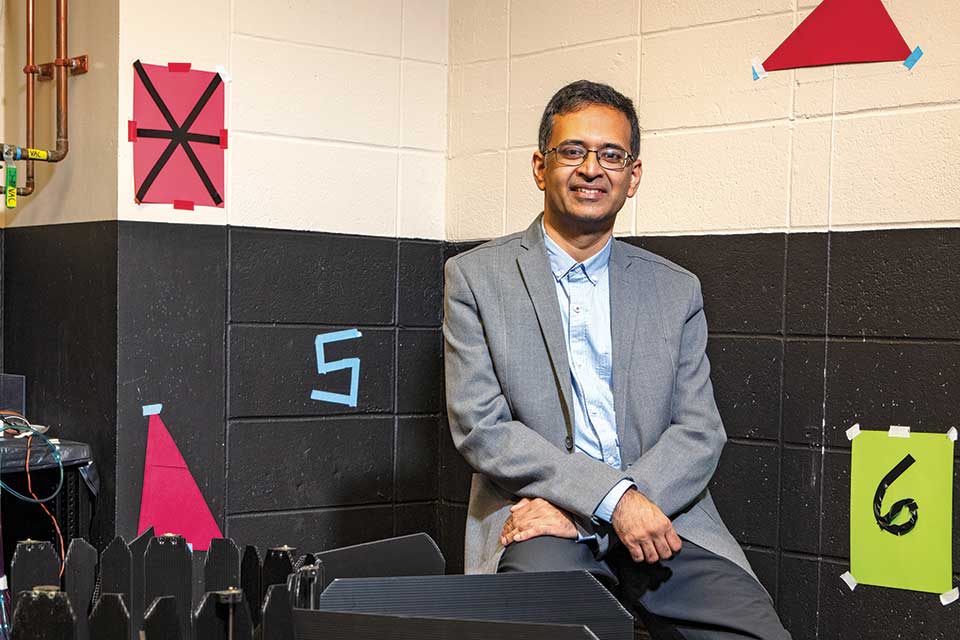
Photo Credit: Jörg Meyer
Watching the brain at work
The basics of memory storage are fairly easy to grasp, at least the way neuroscientist Shantanu Jadhav describes them. At a fundamental level, he says, a single memory is just a discrete chain, or “circuit,” of neurons in the brain, each connected to the next in a unique pattern. When active, these cells pass bioelectrical signals down the line to one another like a tiny mental bucket brigade.
As with all things in neuroscience, however, this gets exponentially more complex as soon as you look under the hood. Although the number of neurons in the brain is finite, the connections they can make among themselves are nearly endless. A single neuron can sprout several long structures called dendrites, thin tendrils that stretch out to meet other neurons in thousands of connections elsewhere in the brain. Through all these tiny pathways, a few dozen cells can be connected in countless different ways, with each new sequence storing a unique memory.
“Basically, you’ve got an ensemble of neurons forming connections to one another in order to store a piece of information,” Jadhav says. “That is the key to encoding memory.”
An associate professor of psychology, Jadhav studies a region of the brain called the hippocampus, a pair of C-shaped fingers of tissue nestled in the center of the cranium. Countless memory circuits start in these structures and meander into other parts of the brain, like a giant railway hub with lines radiating out across the countryside.
The fact that this region of the brain is so central to memory is no surprise, says Jadhav. All our sensory input, everything that happens to us from moment to moment, is constantly being funneled into the hippocampus, where it is processed and temporarily stored. These recorded experiences, called episodic memories, let the brain remember what happened at a specific place or time. It’s how you know what you ate for breakfast, where you left your keys, what grandma’s house smelled like, or what you passed on your way to work today.
We experience life as a perpetual stream of events. The hippocampus helps filter out the salient events, determining which memories to keep, where they’ll live in the brain, and how long they’ll stay there. It acts as an index for the mind, a master librarian that can find which networks of neurons correspond to a specific memory.
“Think of it as a search engine like Google,” Jadhav says. “You need the hippocampus to index where a memory is stored so everything can be retrieved and reactivated.”
At the moment, though, scientists don’t fully understand how this retrieval actually occurs.
Jadhav is working to solve this problem in the lab. He starts by implanting an array of electrodes over the hippocampus of a lab rodent, then placing the animal into a three-pronged maze shaped like the letter E. At the end of each corridor, he offers the rat a small squirt of evaporated milk as a reward, then lets it explore its new home freely.
As it does, he records its neural activity in detail. When the rat reaches the location of each reward, specific neurons called place cells fire repeatedly, encoding and storing a mental map of the maze in its brain.
“If the animal goes from point A to point B, there might be a unique sequence of, say, 50 cells that fire,” Jadhav says. “When it runs to another location, from point B to point C, you get another unique sequence of 50 cells that marks that location.”
If the rodent later returns to a location, this same set of place cells fires again, letting the animal recognize its surroundings.
This particular observation isn’t anything new, Jadhav notes. Neuroscientists have known about place cells and their corresponding neural circuits for years. But his lab is starting to reveal new and unexpected ways in which these circuits are reactivated.
In one recent study, Jadhav found a phenomenon known as “sharp-wave ripples” might be involved in recalling memories. Normally, when the brain is sleeping, these electrical signals course forward and backward through existing memory circuits in milliseconds, making connections between neurons stronger and stabilizing the memory for the long term.
Yet these same signals also begin to fire when the rat is awake, Jadhav found, meaning they could help regulate how a memory is replayed. When a rat encountered a reward in Jadhav’s maze, for example, neurons that represented places it had already visited lit up with activity, carrying sharp-wave ripple sequences in the hippocampus backward in these circuits. Likewise, when the rat was deciding where to go next, Jadhav could detect sharp-wave ripple sequences in the hippocampus traveling in the opposite direction, firing outward on a different chain of neurons.
This finding makes intuitive sense, he says. When a rat reviews the path it just took, the actions closest to the reward seem more important than actions taken farther away, so the rat’s brain may “play” its place-based neural circuits backward, reinforcing the memory of how it arrived at its current location. Likewise, in deciding its next move, the rat’s brain may instantly preview other paths it could take by firing sharp-wave ripples into those circuits.
In rodents, Jadhav says, the presence of sharp-wave ripple sequences in specific place cells is so predictable he can actually use their appearance to tell where a rat will go next, even when it has multiple options.
It’s almost, almost, as if he can read the rat’s mind.
Jadhav, however, doesn’t co-sign this interpretation. “That’s a bit too strong of a way to put it,” he says, laughing. “We say we’re reading the brain’s map. We know how the brain encodes a location and how it reactivates sequences of neurons to recall it. If you record the activity of a large enough sample of neurons, you can decode it to tell the animal’s real-time position.”
Although Jadhav is gaining an incredibly detailed picture of how specific memory circuits work, his research stops short of answering another major set of underlying questions: How do these neurons actually encode information in the first place? How do they become part of a unique, specific sequence? In short, how do they know to become part of a memory?
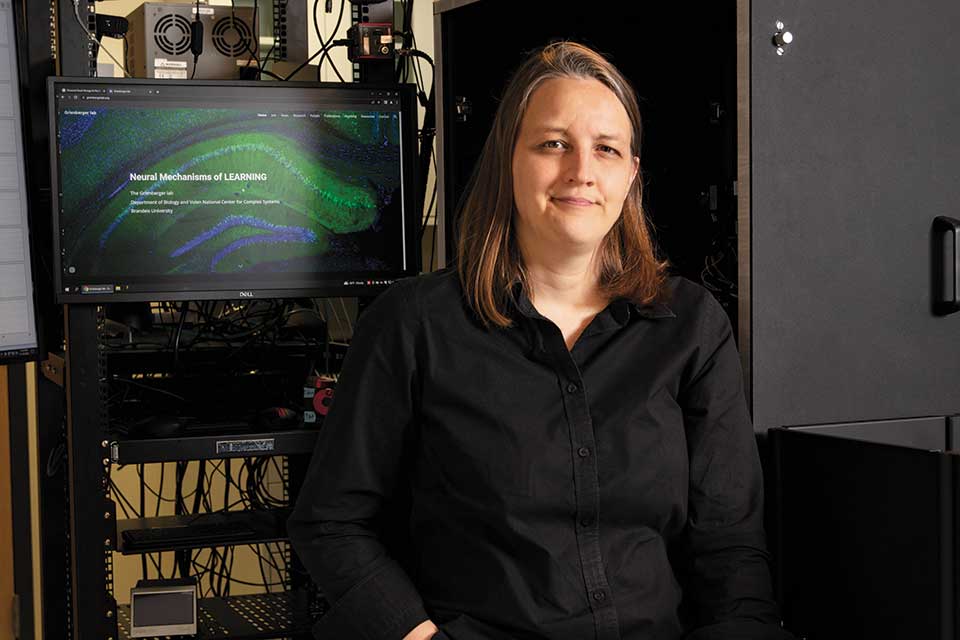
Photo Credit: Jörg Meyer
The secret life of neurons
Answers to these mysteries may emerge in a room just down the hall from Jadhav’s office.
Inside, dim red ceiling lights reveal a black lab bench that dominates the space. A metal rack filled with computer screens and other devices, stretching from floor to ceiling, crowds one side. Just behind that, in the center of the table, a black cloth hood covers a specially built treadmill for lab mice, a contraption that looks like a high-tech belt sander.
This strange room is where Christine Grienberger, assistant professor of biology, chips away at understanding the inner workings of neurons. Like Jadhav, she starts with rodents. The mice are light brown, calm, and curious, gently sniffing at the edges of their plastic containers. They seem pretty much like their wild brethren, with the exception of the flat metal bracket sticking out of their scalp.
The bracket lets Grienberger see, quite literally, inside each animal’s mind. The center of the device, which is surgically attached to the mouse’s skull, holds a tiny glass window that reveals the surface of its active brain. By placing the animal on the specialized treadmill — which secures the mouse directly under a powerful microscope — Grienberger can examine what’s happening to a mouse’s brain as the mouse runs.
The treadmill’s rotating belt, about 2 inches wide and 3 feet long, is split into distinctly textured sections. On one of them, thin plastic bristles stick straight up like grassy tendrils; on another, tiny patches of rough sandpaper dot its surface. On the third and final section, small bits of black Velcro are glued in place. As the belt turns, the mouse’s sensitive paws pick up on these textural changes, and its brain processes them as unique locations. Now I’m in the grass. Now the rocks. The moss. The cycle repeats hundreds of times.
In one of the three “places,” Grienberger gives the mouse a drink of sugar water — a reward for its efforts — and uses her highly sensitive microscope to watch what happens in its brain. Thanks to a special fluorescent dye she injects into the mouse, each neuron gives off a tiny amount of light as it fires. The enlarged images the microscope records are mesmerizing: Through the window in the rodent’s skull, active neurons flash on and fade out slowly over a few seconds, like a swarm of lightning bugs on a summer evening.
Using this method, Grienberger homes in on the specific neurons the mouse’s brain uses to encode a memory of each treadmill section and can then measure any changes that occur when the rodent gets its reward.
“We can tell exactly where the reward location is being encoded in the brain, because when the mouse gets the sugar water, a higher number of place cells become active,” she says. Something in the mouse’s brain seems to reinforce place cells in response to a reward, making the location stand out in the mouse’s memory.
To figure out how this happens, Grienberger singles out one of the glowing neurons and taps into it with a minuscule electrode. This method, called “whole cell recording,” lets her measure the cell’s activity in real time, tracking in exceptional detail the bioelectrical signals that pass through it.
She’s found that if the animal gets a reward at the same location on the treadmill each time, this neuron receives a unique new signal. A region of the brain called the entorhinal cortex (which sits next to the hippocampus) sends the cell an extended pulse of bioelectricity called a “plateau potential,” which lasts far longer than the flickering signals from other sources. This pulse seems to act as a kind of control signal, triggering neurons to suddenly become place cells.
“It essentially tells a neuron, ‘Remember this,’” Grienberger says. “What’s especially interesting is it takes only one exposure to an event or an experience to drive it; a neuron is hit with this one plateau potential, and then a place is encoded in the brain. Unlike other forms of memory like facts and figures, you don’t need hundreds of repetitions to remember it.”
Although the exact way this process works is still a mystery, Grienberger’s research is already paving the way for future research in both the scientific and the clinical realms. With its single-cell focus, it’s taking our understanding of the brain ever deeper and helping provide additional context on the brain to other scientists, including Grienberger’s colleagues at Brandeis.
Some of these researchers are drilling down even further into the brain than Grienberger, looking at the biochemistry of each cell. There, at the smallest and most basic level, might lie the crux of what makes memory possible.
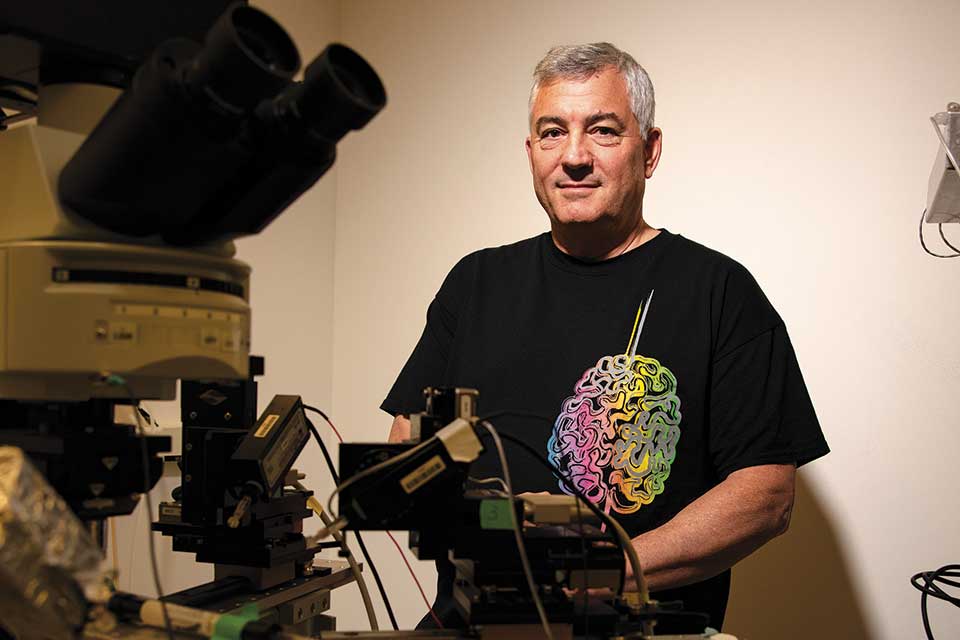
Photo Credit: Jörg Meyer
A strong brain, even under threat
Sacha Nelson speaks slowly and deliberately, with the air of someone who thinks carefully about every word. It may be out of necessity. To accurately describe something as complex as the human brain to a lay audience, choosing the right words is critical. As Mark Twain put it, they’re the difference between the lightning bug and the lightning — or, maybe more to the point, the chaotic electrical storms that flow through neurons as memories are created and recalled.
When each neuron interacts with another in a specific sequence, Nelson explains, connection points between them called synapses start to multiply, and more robust bioelectrical signals flash through them. Once this occurs, a neural sequence is locked into long-term memory.
Nelson, the Gyula and Katica Tauber Professor of Life Science and the neuroscience program chair, studies how these alterations happen. His research focuses on a process called “homeostatic plasticity,” the brain’s ability to change the strength of its cells’ inputs and outputs at will. If it’s working correctly, this process acts as a master volume knob for neural activity: It lets cells grow just enough synapses to strengthen and preserve a memory yet stop before the cell is overwhelmed by incoming electrical signals.
“It’s thought that the memory is really in the pattern of which synapses are stronger and which synapses are weaker,” Nelson says. “One cell might have 10,000 synapses. And one set of synapses might be, say, three times as strong as another set of synapses, but it’s important the sum total of all the synapses not be too strong or too weak.”
Maintaining the right balance throughout the brain is critical. If a cell grows too many new synapses, it can be flooded with electrical energy, maxing out its bandwidth and sending uncontrolled signals to the next cell down the line, causing seizures.
It’s a conundrum. If retaining a memory is a matter of strengthening synapses between cells, why doesn’t the brain hit a saturation point? We can continue to form new memories and learn new things our entire lives. So how does the brain keep things under control?
Nelson thinks it comes down to a complex brew of biochemicals within each neuron. The main way brain cells communicate with one another is by releasing the amino acid glutamate, which latches onto specific receptors on its neighboring cell. This process activates a signal in the receiving cell that lets calcium ions flow inside it, setting a suite of biochemical processes into motion.
Transcription factors, the enzymes that create proteins from RNA, immediately go to work. This process kicks off other transcription factors, which, in turn, activate other signaling molecules. These molecules change the activity of proteins, which alter how the neuron functions. It’s a complex cascade.
“This really is the beginning of the change in synaptic strength,” Nelson says. “The key signal is a combination of a neuron’s own activity and the activity of another cell that’s providing input to it. We know some of the transcription factors that are important at the beginning of the process, and we know some of the proteins that change the behavior of the neuron at the end of it. But we really are just starting to find the things that happen in the middle.”
It’s a hairy problem, but Nelson is confident it’ll be solved in the not-too-distant future. Research on homeostatic plasticity is already helping to reveal how the brain keeps itself running even when under threat. Its ability to change and redirect synapses at will, Nelson posits, may keep our minds going strong after injury or disease has inflicted damage.
He says, “It’s clear the nervous system has an enormous capacity to deal with loss of function for a very long time before you completely decompensate and become demented. I think homeostatic plasticity has a lot to do with that. Let’s say that, all of a sudden, you’re not operating on six cylinders but five, or even four. If you can dynamically adjust your car’s powertrain so it still moves, you can maintain its function even when the engine is damaged.”
A deeper understanding of how single cells change in the brain could also help protect the organ from neurological diseases. As disorders like Alzheimer’s or dementia progress, the signals neurons routinely send to one another are slowly choked off, scrambling the way the brain makes and recalls memories.
Using the data Brandeis researchers are generating, it may one day be possible to artificially manipulate memory circuits in Alzheimer’s patients, helping to reinforce and preserve memory at will — if, that is, scientists can tease out the precise ways the disease actually works.
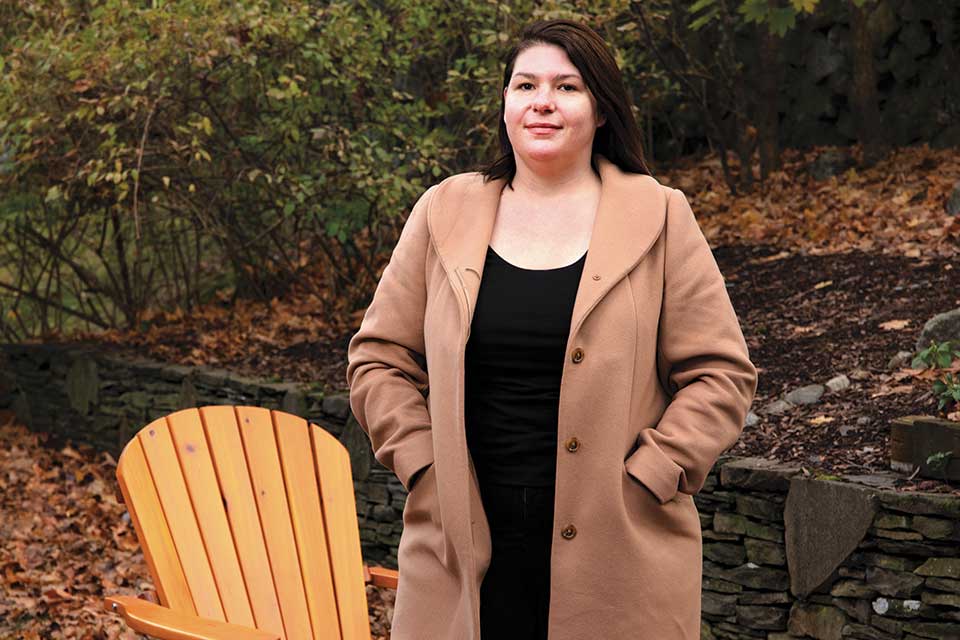
Photo Credit: Jörg Meyer
Detecting disease before it starts
One of the primary physical indicators of Alzheimer’s is abnormal proteins that cluster throughout the brain. Generally speaking, two forms of these clusters — tau tangles and amyloid plaques — spread throughout a patient’s gray matter, destroying both the brain’s circuits and the cells that create them.
Whether tau or amyloid pathology is the main cause of the disease is a matter of debate. In either case, the outcome is the same. Patients are progressively robbed of existing memories and left unable to create new ones. As the disease advances, they’re doomed to live in an ever-thickening fog, struggling to regulate emotions, make simple decisions, and differentiate recent events from the distant past.
Neurobiologist Anne Berry, an assistant professor of psychology, is working to understand how these diseases of memory break down pathways in the brain and how this degeneration might be prevented. Rather than looking at the cellular level, Berry is taking a broader view. She focuses on neurotransmitters, chemicals in the brain that enable communication between neurons and help regulate their behavior. Two of these chemicals, she says, could play a major role in preserving memory.
One is called norepinephrine, a molecule that’s involved in a long list of cognitive processes, from attention, to threat response, to sleep-wake cycles. It also has protective functions, helping to keep neuronal circuits going strong even in the presence of pathology. The other neurotransmitter, called dopamine, is released in the brain when we encounter surprising rewards, like the sweet treats Jadhav and Grienberger give their rodents during experiments. It also helps protect the brain by calming overactive neurons.
In patients with Alzheimer’s, the hippocampus is strangely overactive, with entire clusters of neurons firing rapidly. This activity is detrimental to the brain. As the cells in the hippocampus activate more quickly and frequently, they can start to die off, and pathology accumulating inside and around them begins to spread into other parts of the brain.
A $6.2 million grant from the National Institutes of Health is helping Berry examine this through a new multiyear effort called the Brandeis Aging Brain Study. Over the course of the project, she hopes to find a relationship between plaques, neurotransmitters, and memory, using advanced medical imaging techniques like functional magnetic resonance imaging and positron emission tomography. She’ll scan the brains of a diverse cohort of volunteers while they conduct a battery of memory tests, recording how neurotransmitter levels shift with activity. Every three years, these same subjects will return to do the tests and scans again, enabling Berry and her team to identify changes that take place in the brain over time.
“We’re really interested in seeing if we can learn from people’s baseline scans how plaque accumulates, how their dopamine and norepinephrine levels are changing, and how that is predictive of their cognitive function,” Berry says. Another big goal will be understanding why some people are able to have large amounts of plaque in their brains yet retain perfectly normal cognition.
Most of the 400-500 participants in the study are still being recruited. On the basis of early data, Berry already suspects that people with higher levels of dopamine and norepinephrine than their peers may be better able to maintain their cognitive function later in life.
“Even if you have pathological insults like plaques in the brain or if you’re starting to develop Alzheimer’s disease, you may be able to make up for some of those losses by increasing your dopamine or norepinephrine production,” she says. “If we can catch things before they really start, when people are in their 60s, 70s, and even early 80s, we could make a meaningful impact on the trajectory of their brain health.”
Ultimately, this is the goal of all these researchers’ work — to understand memory so we can protect it. Progress may be incremental and slow, but any advancements have an outsized importance for us as humans. It doesn’t matter whether insights come from a human subject in a medical scanner or a single neuron under the microscope. Every new discovery, every step forward, adds to our knowledge of something central to us.
To remember is to exist. The thrill of a first love; the grasp of a child’s tiny hand; the smell of the earth after a heavy rain — all the beauty we see and the awe we feel as we move through the world is nestled in our head. For as long as our mind holds these experiences, they remain frozen in time.
As scientists like Jadhav, Grienberger, Nelson, and Berry move closer to their goal of understanding how our brain maintains our memories, they come ever closer to safeguarding our humanity as well.
About the Author
David Levin is a freelance science writer living in Boston.